I’ve written about neutrinos several times now in this blog. (See posts of 30July2017, 2December 2017 and 6June2018.) Neutrinos are known as ghost elementary particles because they interact so rarely, so weakly with other particles. In fact, although billions of neutrinos are passing through your body every second you’ll be lucky if a single neutrino interacts with you during your entire life.
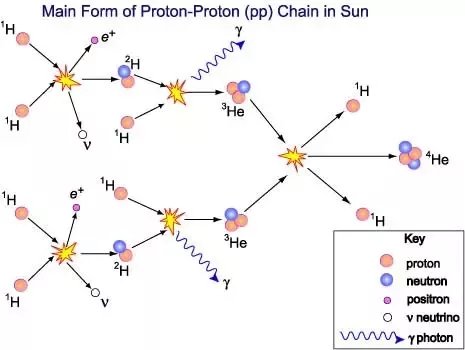
Most of those neutrinos come from the fusion processes that produce the energy of our Sun but there are also anti-neutrinos whizzing about that mostly come from the decay of radioactive elements in the ground along with the fission reactions in nuclear reactors. I’ve often considered it amusing that fusion, which takes lighter elements and combines them into heavier elements, produces a lot of neutrinos while fission and nuclear decay, which break down heavy elements, produce mainly anti-neutrinos.

For almost fifty years now scientists have been using neutrinos in order to study our Sun, and learned a great deal about neutrinos in the process. Neutrino detectors consist of large containers of water that capture the neutrinos while photomultiplier tubes that line the inner walls of the container detect the faint light produced by those captures. The detectors themselves are buried deep within mine shafts in order to minimize the interference caused by cosmic rays. These ‘neutrino telescopes’ detect no more than a handful of neutrinos a day but those few particles have lead to many important discoveries.
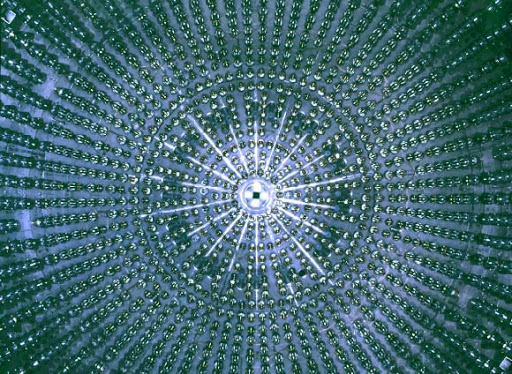
Now another neutrino observatory is using anti-neutrinos, known as geoneutrinos to study the interior of the Earth. The Borexino detector, located at the Laboratori Nazionali del Gran Sasso in Italy and containing some 1000 metric tons of water has been in operation now since 2007. Buried 1400 meters beneath the Earth’s surface in the Gran Sasso massif near Rome, Borexino has succeeded in capturing 53 geoneutrino events. (It’s worth noting that during that time the detector was also capturing Solar neutrinos.)
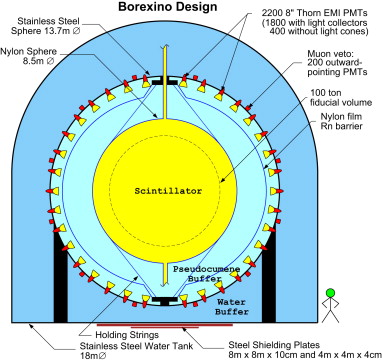
And those 53 anti-neutrinos were enough to allow the theoreticians to answer a question that has long perplexed geologists and geophysicists, how much of the Earth’s internal heat is being generated by the decay of radioactive elements? Thanks to the data provided by Borexino we now know, with an 85% confidence level, that just about half of the heat in our planet’s core comes from nuclear decay. In fact the data obtained by Borexino has even allowed physicists to estimate the amounts of the radioactive elements Uranium and Thorium remaining inside the Earth.
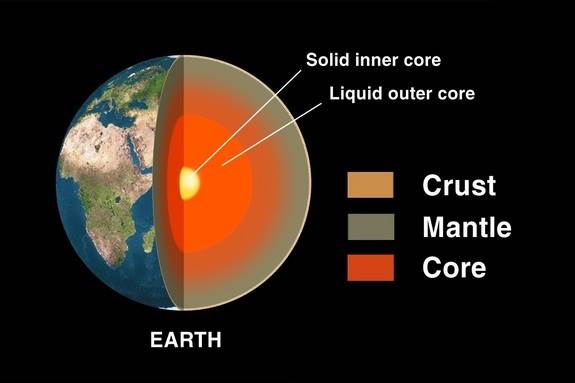
Borexino will continue to capture geoneutrinos, and each new detection will increase the accuracy of the results. There are also plans to build a larger detector at Gran Sasso so in the years to come geoneutrinos may tell us even more about the makeup of the interior of the Earth.
Now if you think that calculating the energy generated in the Earth’s core based on just 53 detections of elementary particles is pretty amazing well there are a group of physicists attached to the Antarctic Impulsive Transient Antenna (ANITA) experiment in Antarctica who think that the detection of three particles could overturn the standard model of particle physics.
ANITA is a completely different sort of neutrino detector than Borexino, but one that is only sensitive to the very highest energy neutrinos, those with energies far greater than that of neutrinos coming from either the Earth or Sun. ANITA is intended to study the neutrinos associated with high-energy cosmic rays from quasars or black holes or supernovas.
The way ANITA detects these high-energy neutrinos is that while in space the neutrinos are moving at almost, but not quite the speed of light in a vacuum as they pass through the Antarctic ice they are actually moving faster than the speed of light in ice! Such particles will give off a kind of radiation known as Askaryan radiation until their speed is reduced below that of light, or they leave the ice. The radiation that these high-energy neutrinos give off happens to be in the microwave region of the electro-magnetic spectrum, a type of radiation that we humans are very good at detecting. Your cellphones operate in the microwave region for example.


Funded by NASA, the ANITA experiment therefore consists of an array of 40 microwave antennas that are lifted into the stratosphere over the Antarctic by a helium balloon in order to maximize their coverage area. Operating on an every other year basis ANITA has now flown four times and detected numerous signals associated with high-energy neutrinos that have taught physicists a great deal about the neutrino component of Ultra High Energy Cosmic Rays (UHECR).

Three of those detections however seem to come directly up from beneath the detector, as if they’ve come through the entire Earth, something high-energy neutrinos should not be able to do. Remember the whole time that they’re passing through solid material the neutrinos are giving off Askaryan radiation.
So if these three particles weren’t the sort of neutrinos that physicists are familiar with, what were they? Some new form of neutrino? Or an entirely new type of particle beyond the Standard Model? Three detections do not give enough evidence for a definitive answer but ANITA is only one of a number of experiments that are giving indications of physics beyond the Standard Model.
Further flights with ANITA are planned, as are other experiments designed to give a clearer picture of what these strange particles might be. Physicists have never been happy with the Standard Model, which fails to answer as many questions as it does answer. How long it will take to understand what is beyond the Standard Model, and what experiment will finally succeed in making the breakthrough is anybodies guess. You can be certain however that physicists will keep on looking until they find those answers.