We humans like to place the objects we find into distinct categories, male or female, dog or cat, living or non-living. Nature doesn’t really work that way however, the edges between different classes of objects are often quite fuzzy. Take stars and planets for example, back in my post of 22 September 2021, I discussed an relatively new class of objects called brown dwarfs, objects that are too heavy to be planets, but too light to be stars.
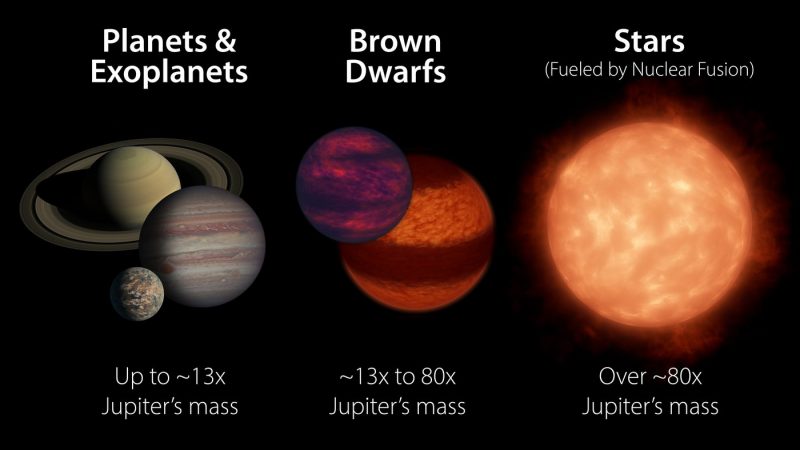
Strictly speaking brown dwarfs do not have enough mass to cause the pressure and temperature at their core to ignite the process of hydrogen fusion. They are larger than planets however and do emit some infrared light because the gasses they are made of continue to collapse due to gravity and that shrinking generates heat.
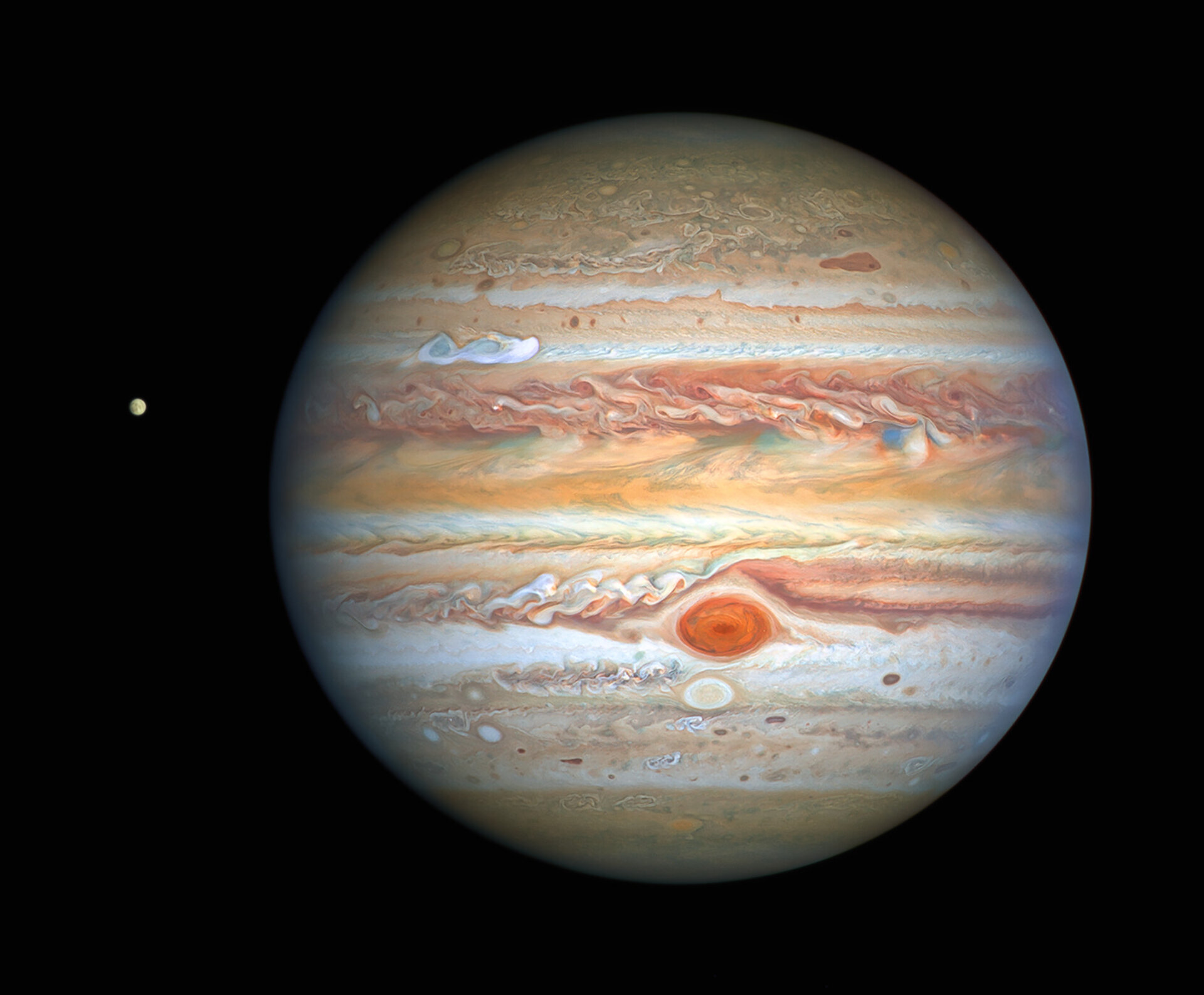
One of the smallest, and coolest brown dwarfs ever discovered is known as WISE J062309.94-045624.6, (I’ll just call it J06 from now on) which is located about 7 light years from our solar system. The size and mass of J06 are only approximately known, its diameter is between 0.95 and 0.65 that of Jupiter while it’s mass is at least four time Jupiter’s, but not more than 44 times. We do have a rather accurate measurement of it’s surface temperature however, around 425ºC making it about as hot as a wood burning fireplace.
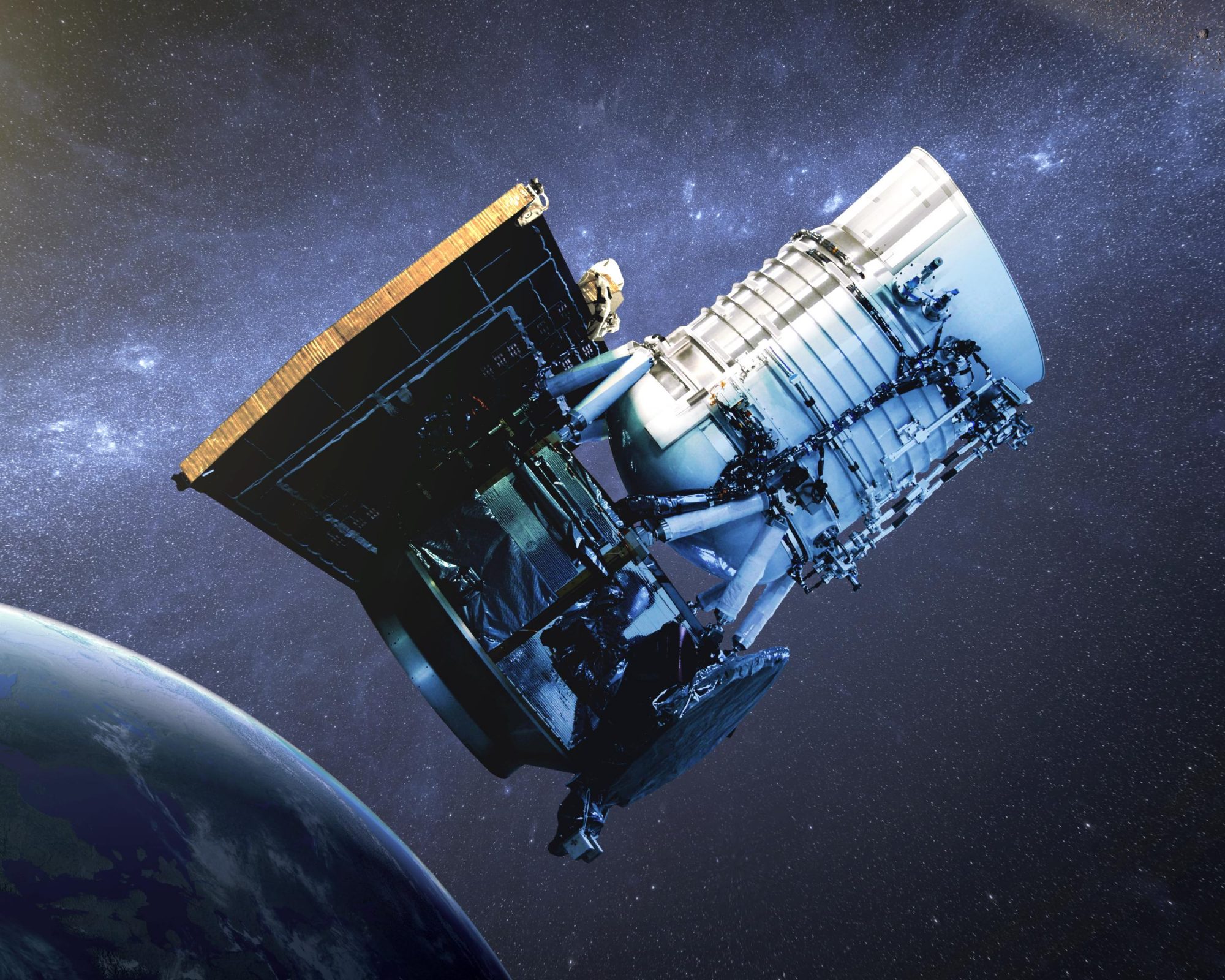
Being so cool it was something of a surprise therefore when observations of J06 by the CSIRO ASKAP radio telescope in Western Australia showed that the dwarf was broadcasting periodically at frequencies between 0.9 and 2.0 Giga-Hertz (That’s between 900 million and 2 billion cycles per second). These observations were later confirmed with the Australia Telescope Compact Array and South Africa’s MeerKAT telescope.
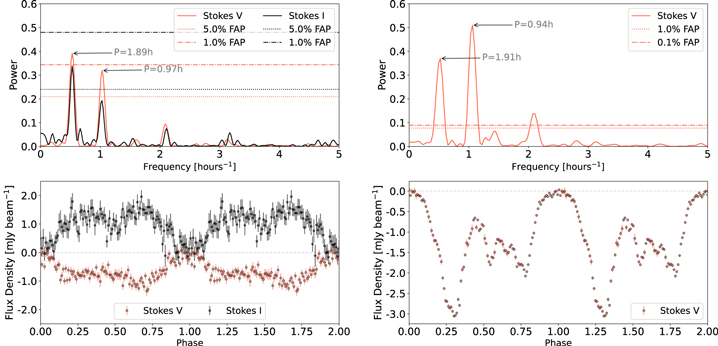
The time period for the radio emissions was found to be about 1.91 hours which is thought to be the time it takes the dwarf to rotate on its axis, its day that is. An analysis of the data from J06 by researchers at the University of Sydney, including lead author Ph.D. candidate Kovi Rose has led to the conclusion that the dwarf possesses a magnetic field of greater than 700 gauss that is generating the radio emissions.
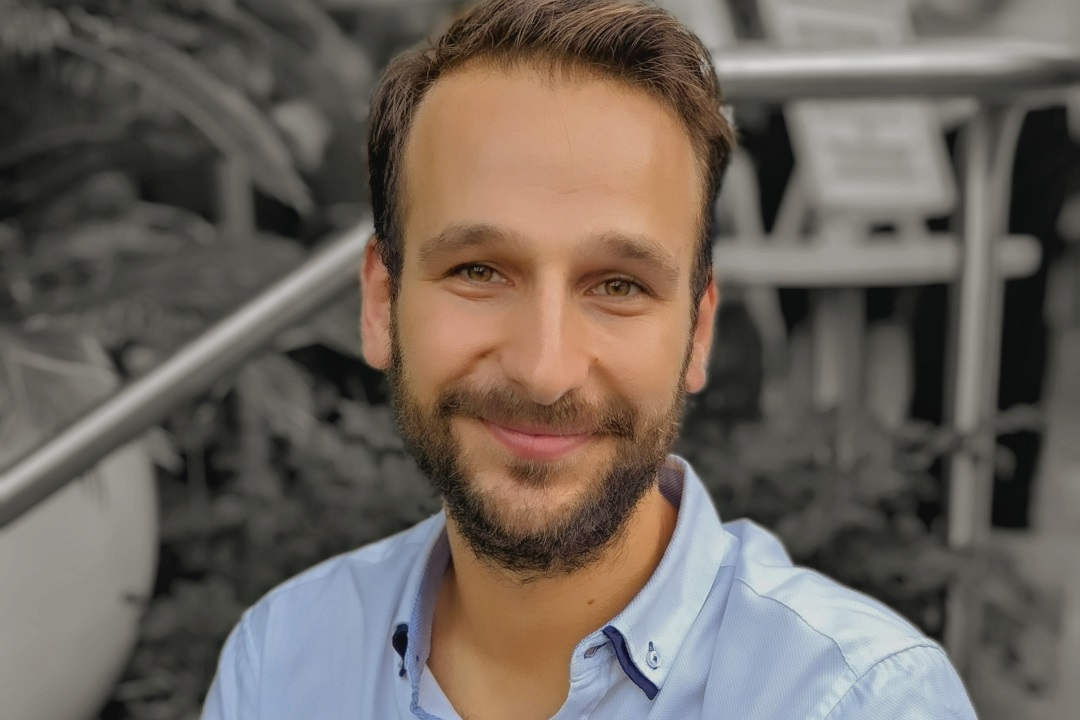
Only a small number of Brown Dwarfs have been discovered so far by astronomers and there is much we don’t know about this class of celestial objects. Only by finding more dwarfs, maybe by using their radio emissions to detect them, can we learn more about these objects.
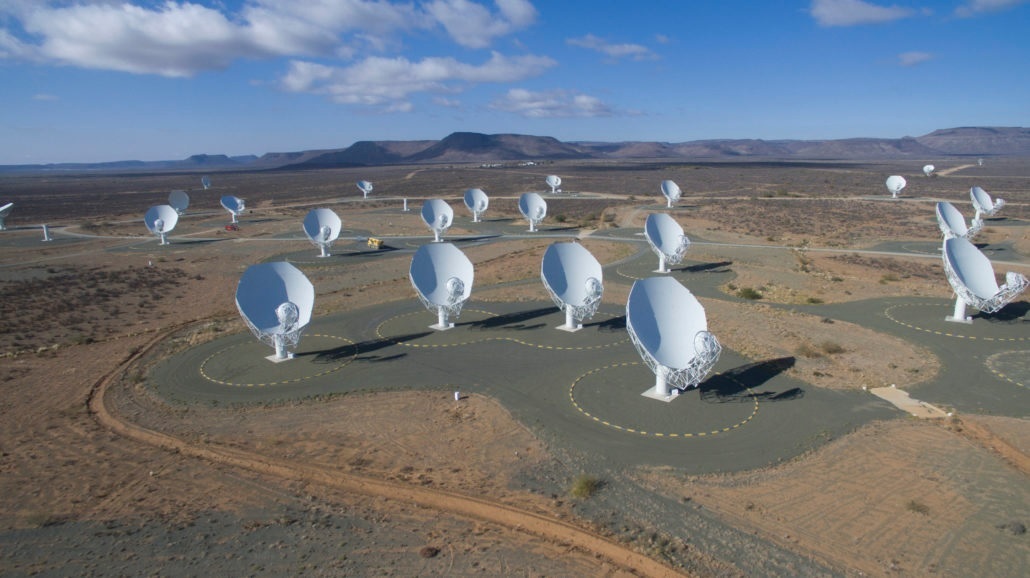
Unlike normal stars, Brown Dwarfs are studied by observing them in the infrared or radio portions of the electro-magnetic (EM) spectrum. One hundred years ago such observations could not have been carried out simply because the instruments needed to detect infrared and radio energy did not exist. Today however astronomers also have instruments that allow them to observe in the Ultra-Violet and X-ray portions of the EM spectra so that we can “see” the Universe in those lights as well.
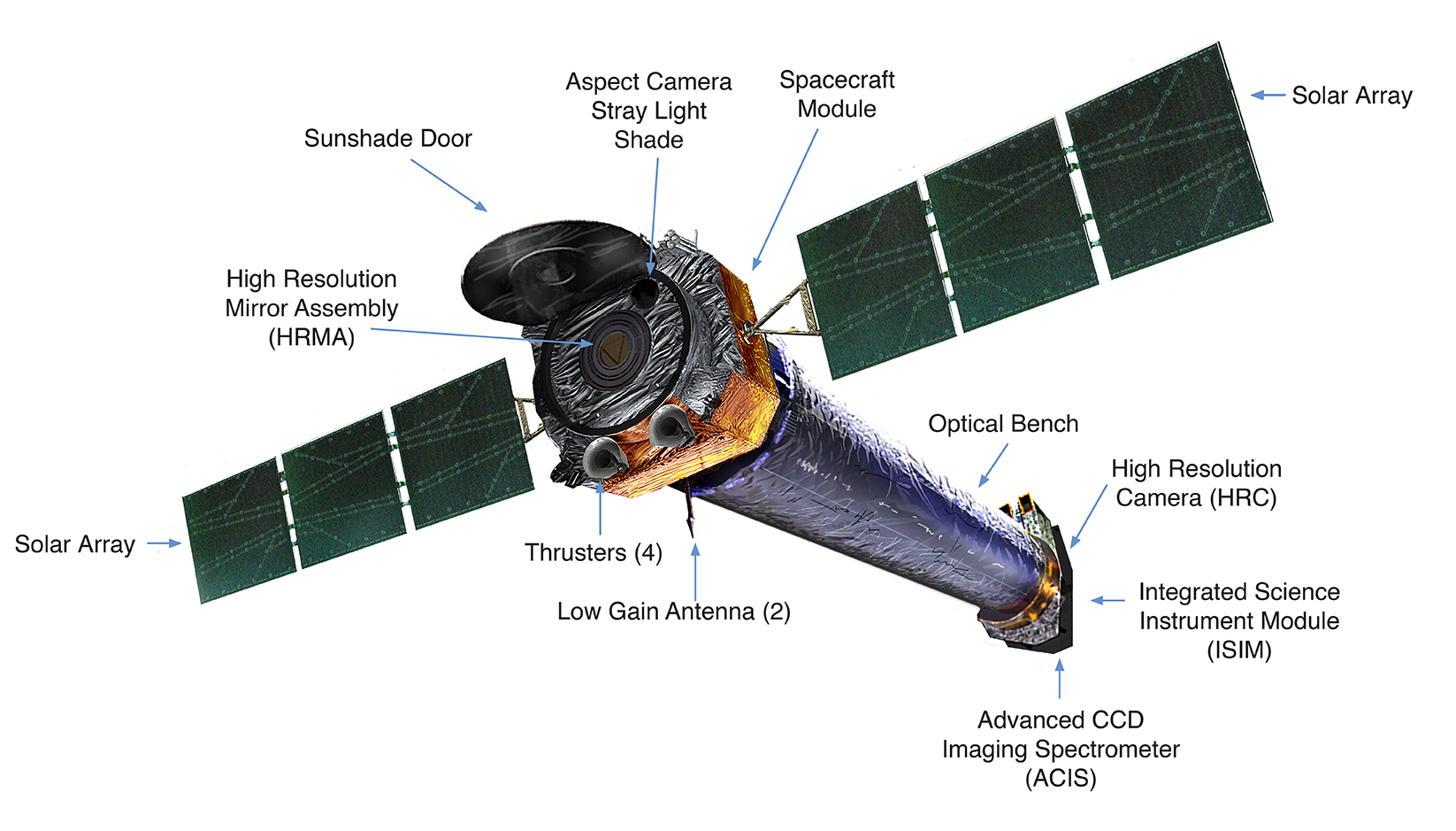
More than that, today astronomers can even make observations of the Universe using Cosmic Ray particles and Gravity Waves, see my posts of 14 June 2017 and 22 October 2017. In fact every time that astronomers have found a new way to observe the Universe, a new form of energy with which to make astronomical studies, they have discovered whole new kinds of celestial objects and learned even more about the objects they already knew.
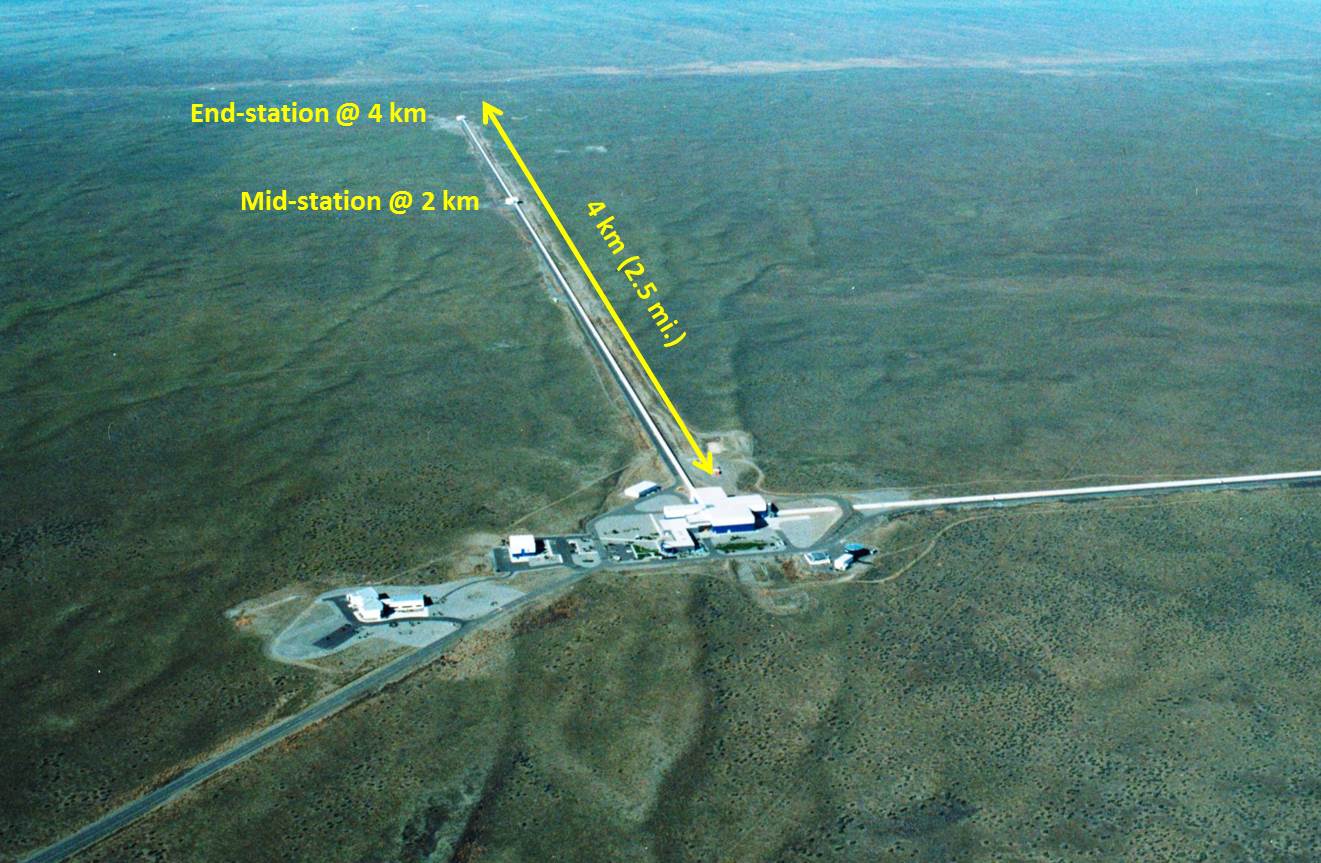
One type of radiation that astronomers that tried for a long time to employ are neutrinos, those ghost like sub-atomic particles that can pass through the entire Earth with hardly any of them interacting. That’s why neutrinos are so hard to use for astronomical observations, you need huge detectors, and lots of time, in order to catch just a few of them.
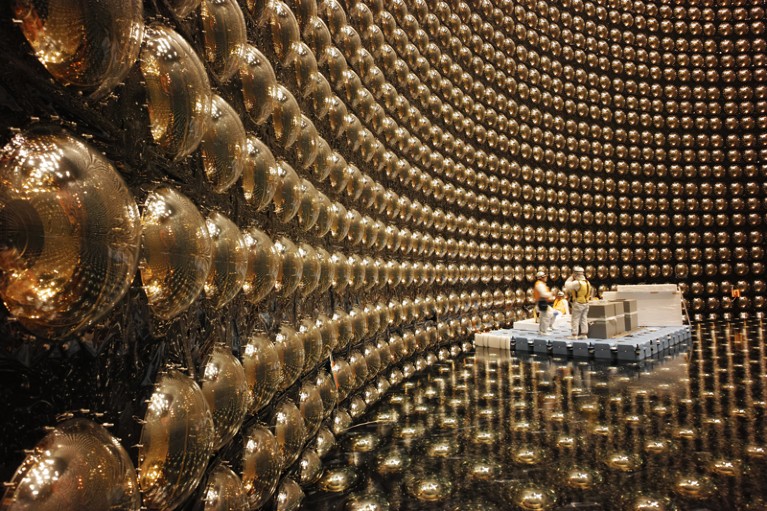
That hasn’t stopped astronomers and astrophysicists from trying to use neutrinos however. The first time was a neutrino detector buried in the Homestake mine in South Dakota that was designed to detect neutrinos produced by the process of hydrogen fusion deep within the Sun. This experiment ran from 1970 to 1994 and taught us a great deal about both the Sun and neutrinos. Then, in 1987 the first supernova in our galaxy for over 300 years was detected and just as astrophysicists had predicted the Sudbury neutrino experiment detected about a dozen neutrinos from the distant event.
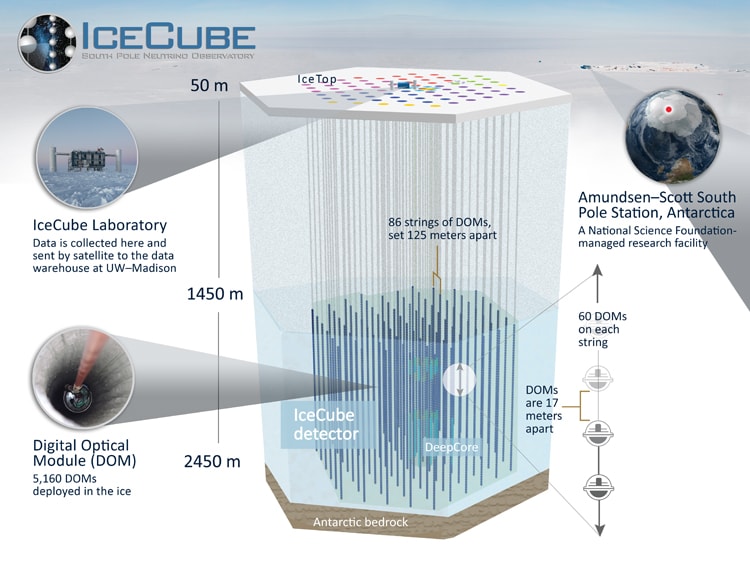
Now astronomers have constructed the largest, in terms of volume, experiment ever in the ice covered continent of Antarctica. The Ice Cube Telescope as it is known uses the fact that when a neutrino does interact with more normal matter it causes the emission of a few photons of light, photons that can travel a considerable distance through the Antarctic ice.
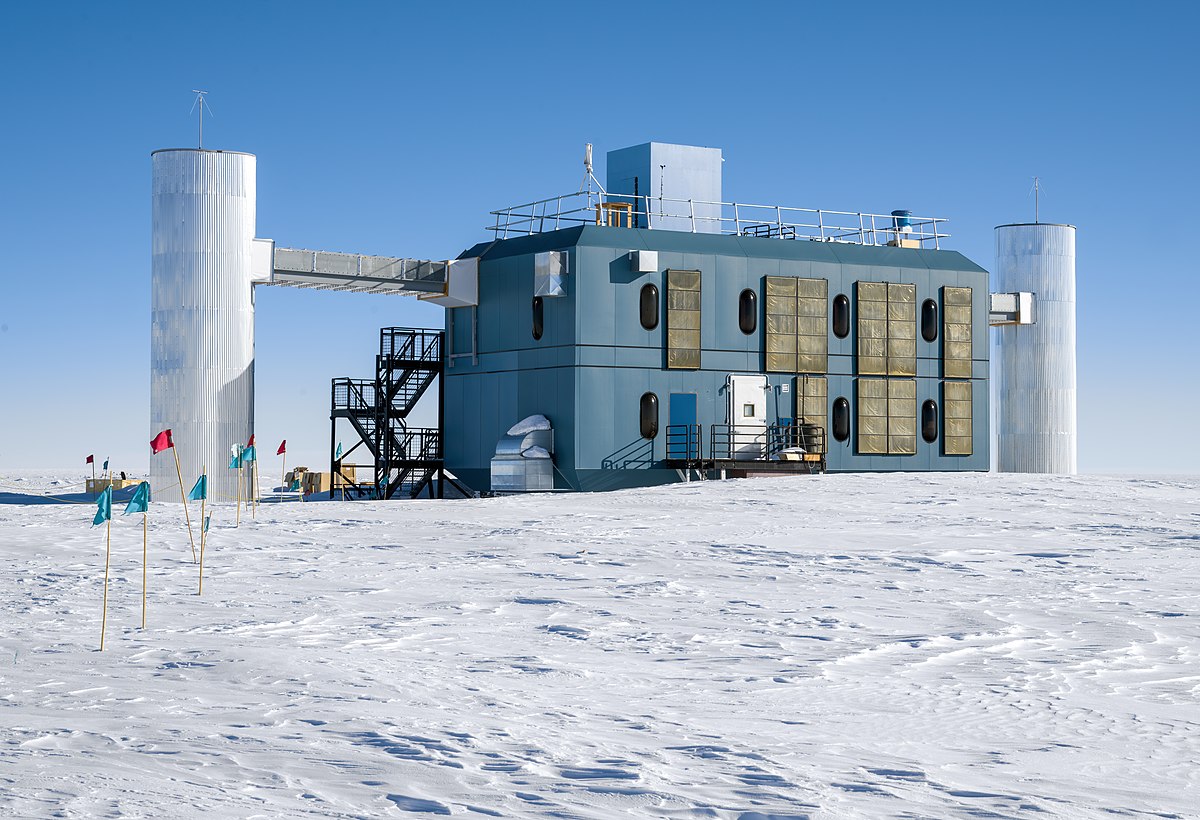
The Ice Cube Telescope was constructed with a full cubic kilometer of glacial ice near the Amundsen-Scott South Pole Station. Drilling holes down into the ice scientists buried over 5,000 light detectors so that they could detect the light generated by any neutrinos that were absorbed in that cubic kilometer of ice. Despite its huge size the Ice Cube detector still only captures a small number of neutrinos every day so, like taking a picture in very low light, in order to form any kind of image a long exposure time was required.
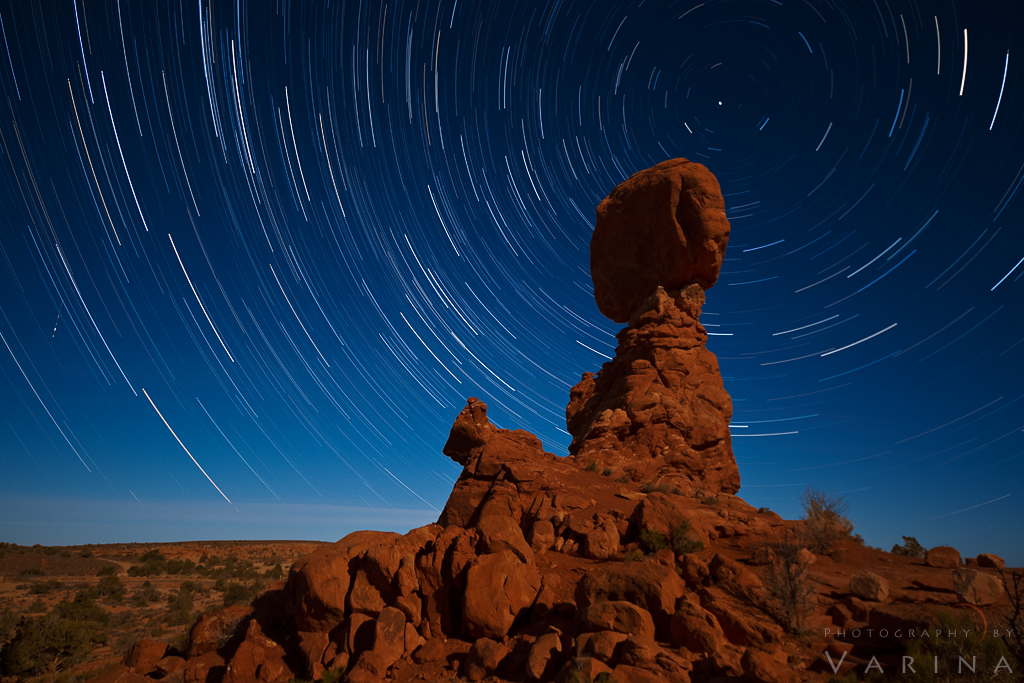
In fact it took over 10 years to collect 60,000 neutrino generated collisions and a special computer algorithm in order to form the first ever neutrino image of our Milky Way galaxy. Researchers from Drexel University’s Department of Physics Naoko Kurahashi Neilson, Associate Professor along with graduate student Steve Sclafani performed the processing that produced the image shown below.
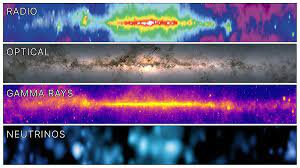
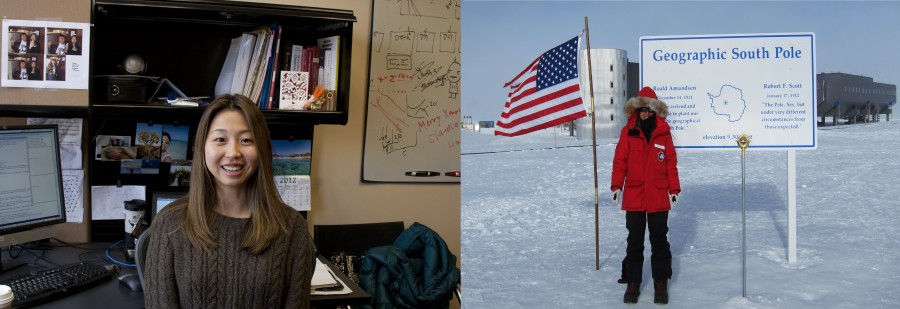
This picture represents the birth of an entirely new kind of astronomy, neutrino astronomy. Right now we can only guess what neutrino images will tell us about the objects we already know about, but more importantly what new kinds of astronomical objects will be discovered using neutrinos.