The Standard Model of particle physics has several problems. For one thing it simply doesn’t contain gravity in any way. Another problem is the masses of all the particles. For example the muon resembles an electron in every respect except its mass, which is 206.84 times that of its cousin. The standard model can’t simply doesn’t explain that ratio or any of the other mass ratios. In fact the whole concept of generations, particles like the electron, muon and tau that behave in the same fashion except for their mass, is a complete mystery at present. Perhaps the biggest problem with the Standard Model however is that it works so well that we have very few clues pointing toward a more comprehensive theory that will answer our questions.
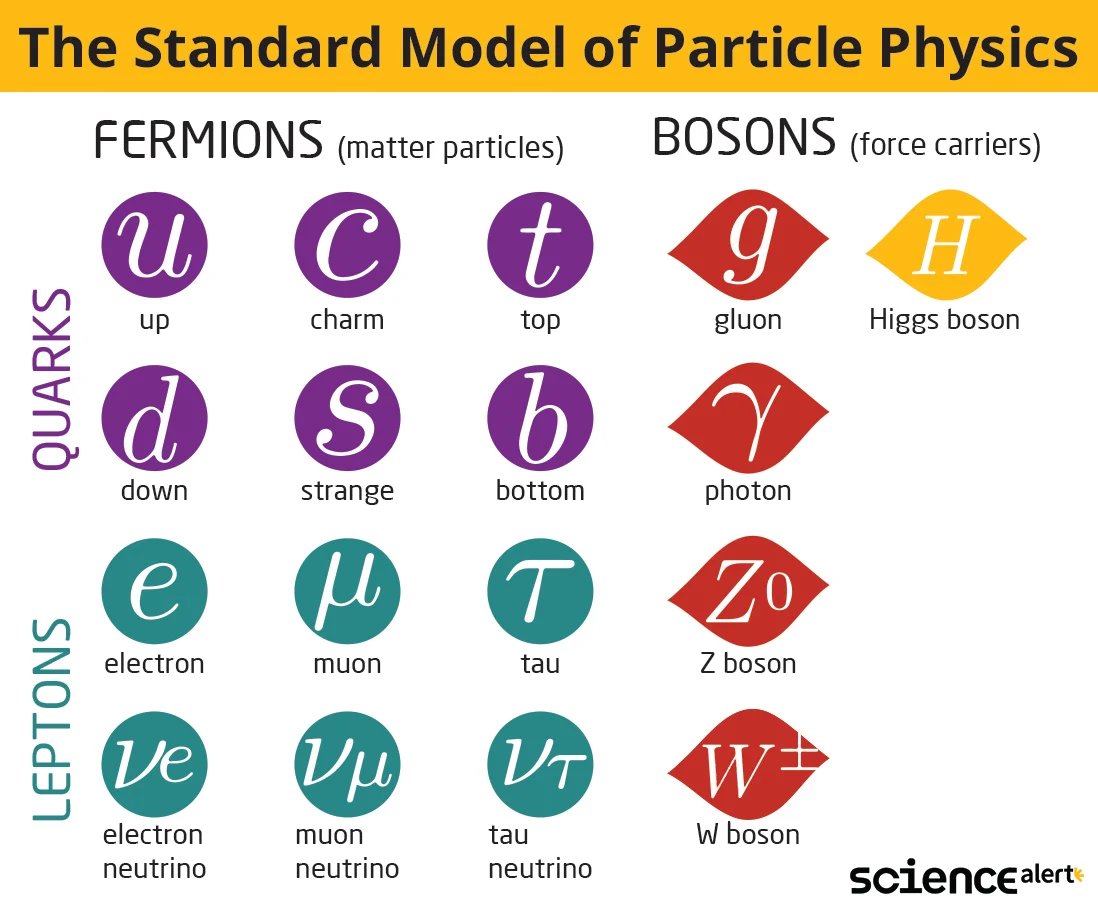
That’s part of the reason why physicists are so busy studying the particle known as the neutrino. These ghost particles have mystified physicists ever since their existence was first predicted by the theoretician Wolfgang Pauli. Pauli proposed the neutrino to explain some discrepancies in the type of radiation known as beta decay.
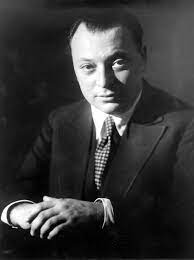
Now in beta decay a neutron splits into a proton and an electron. In the process conservation of the electric charge works out, a neutron is neutral while the positive proton and negative electron still add up to zero. The energy of the proton and electron did not always come out the same however, a violation of conservation of energy. And the spins of the particles were just all wrong as well, again violating conservation of angular momentum.
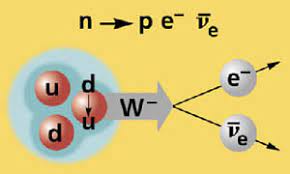
What Pauli proposed was that a third particle, both electrically neutral and with zero rest mass, was emitted at the same time and the experimentalists just hadn’t detected it yet. At first Pauli called his particle the neutron but when the bigger, massive neutron was discovered by James Chadwick, Enrico Fermi then suggested Pauli’s neutral particle be called the neutrino, which is Italian for ‘little neutral one’. Well it took more than twenty years but eventually Pauli’s neutrino was discovered in 1956, and in fact physicists now know that there are three different types of neutrino, one each complimenting the electron, the muon and the tau particles. Again why each generation of electron like particle should have its own neutrino is simply not explained in the Standard Model.
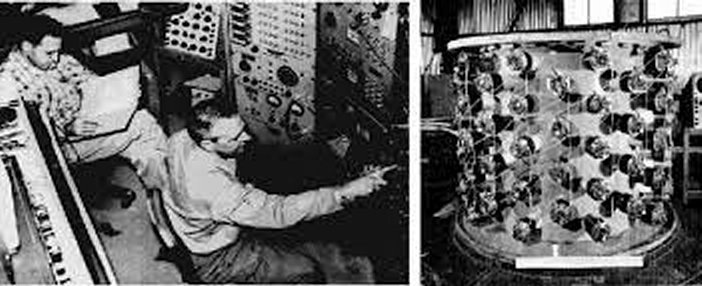
Now neutrinos interact very rarely with other particles, it’s estimated that a neutrino could fly through a light-year of solid lead and still have a 50-50 chance of coming out the other side. At the same time neutrinos are generated in large amounts in nuclear reactions, such as the fusion reaction that powers our own Sun and the other stars. Solar physicists therefore wanted to try to capture as many solar neutrinos as they could hoping to learn about the interior of the Sun from them.
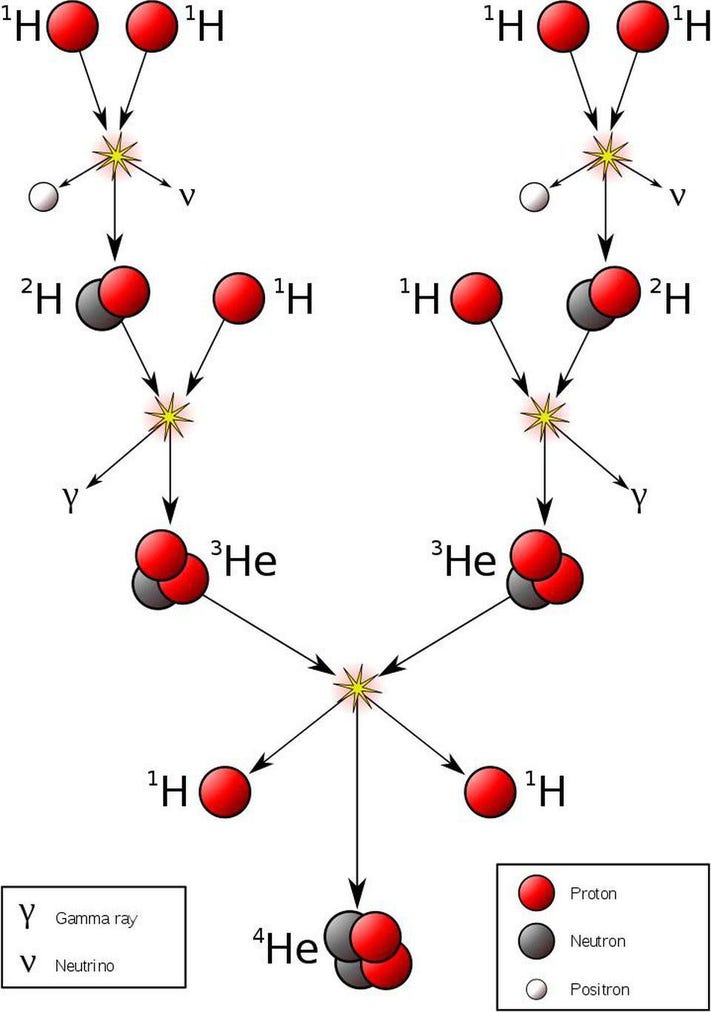
Instead they learned more about neutrinos. The first neutrino telescope was built deep beneath the Earth’s surface at the Homestake Mine in South Dakota in order to eliminate contamination from cosmic rays. What the telescope found was that the number of neutrinos coming from the Sun was exactly one-third the expected number. After wondering for some time if something was wrong with their theories of solar fusion, or maybe something was actually wrong with the Sun the physicists eventually found that the three types of neutrino oscillate, that is they change from one type to another over time. The neutrinos generated in the Sun are all electron neutrinos but by the time they reach Earth two-thirds have changed to muon or tau neutrinos.
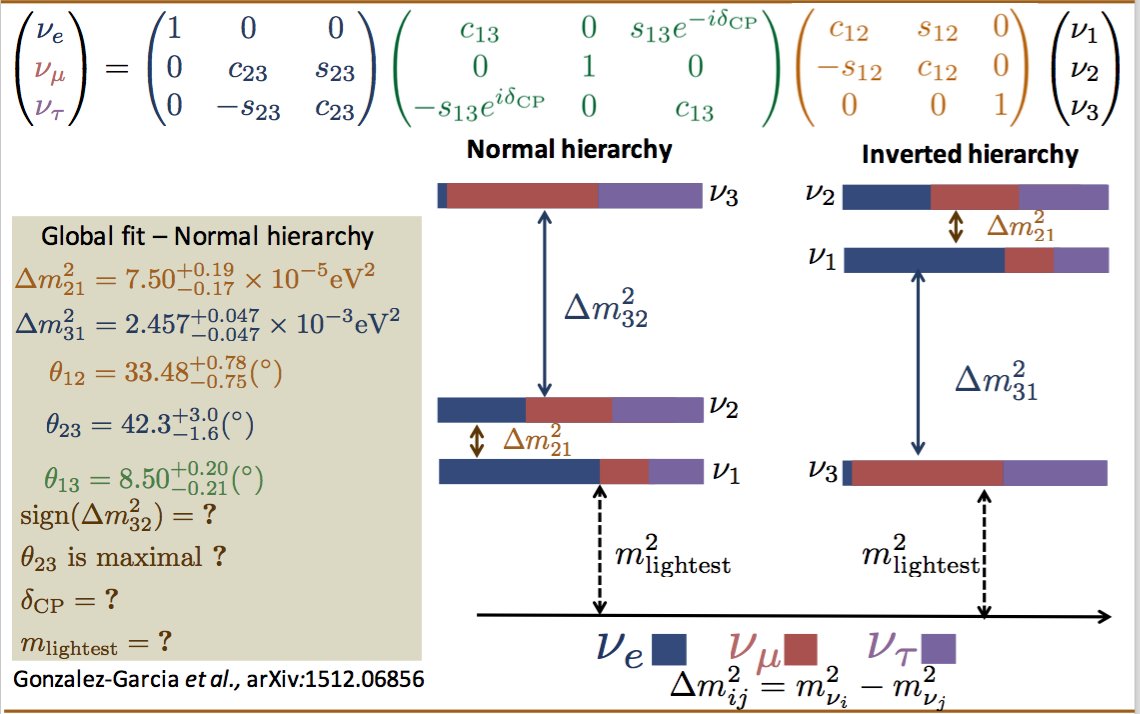
Which means that neutrinos must have a rest mass because particles with zero rest mass move at the speed of light and according to Einstein’s theory of relativity time does not pass for anything moving at the speed of light. So the questions now are, just what is the mass of a neutrino and can we learn a clue from that about the masses of all the particles.
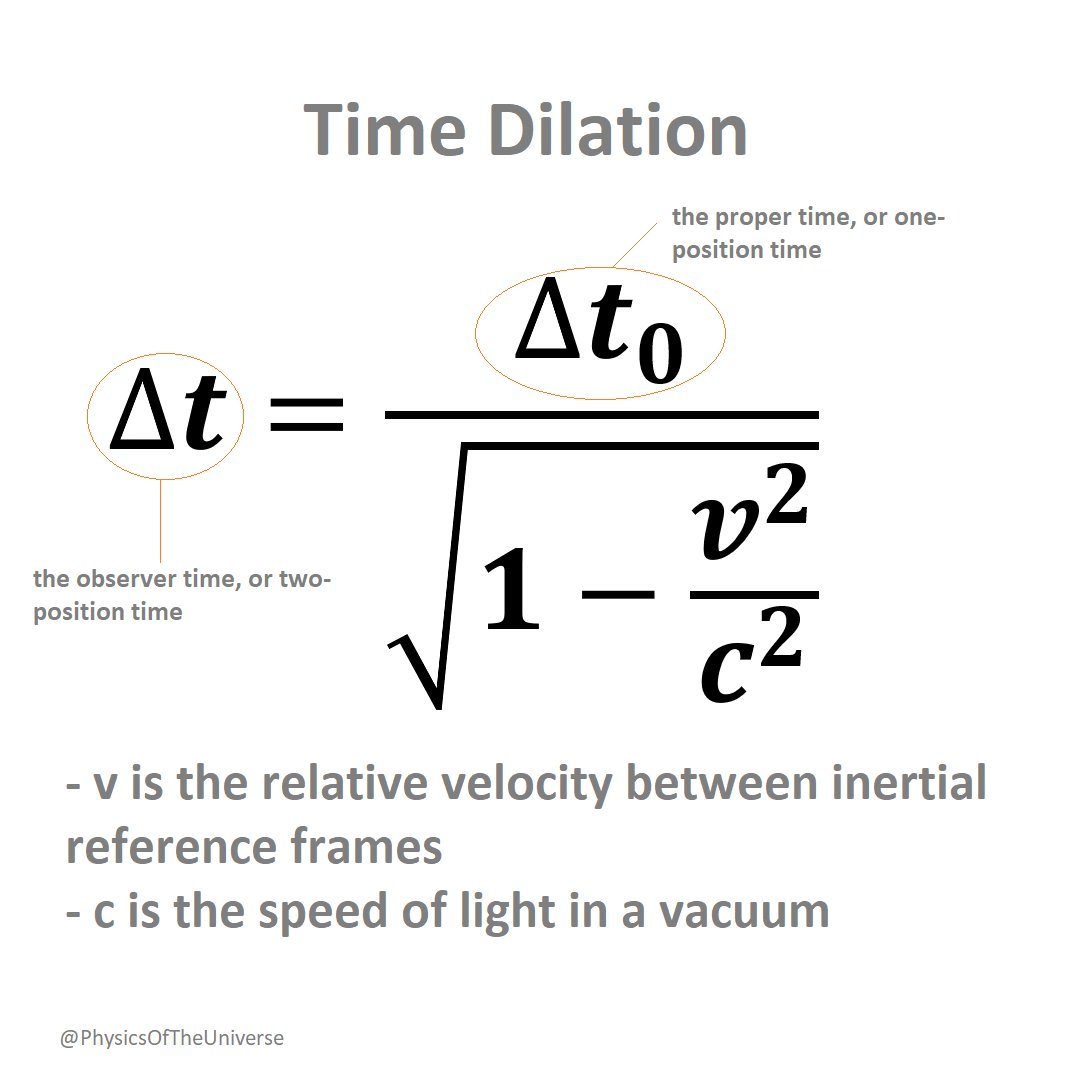
That’s the purpose of the KATRIN experiment at Karlsruhe Institute of Technology in Germany. KATRIN is trying to measure the mass of a neutrino by making the most precise measurements ever of beta decay, the original interaction for which Pauli first proposed the neutrino. Think about it, if the energy of a neutron gets shared in varying amounts between a proton, electron and a neutrino the minimum amount of energy the neutrino can get is its rest mass. So if you measure thousands or better still millions of neutron decays the maximum energy of the proton and electron taken together and subtracted from that of the neutron, is the rest mass of the neutrino. Easier said than done, remember we’re talking about sub-atomic particles here and previous experiments have already concluded that the neutrino rest mass is less than 1/100,000th that of the electron.
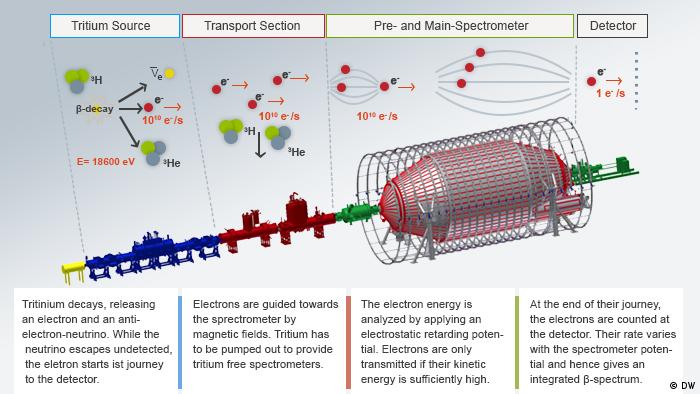
Let me take a moment here to mention the units by which particle physicists measure mass. Remembering Einstein’s most famous equation E=Mc2 physicists like to turn that equation around to get m=E/c2. So to describe the mass of elementary particles physicists use a measure of energy known as the electron-volt, the energy an electron will gain by accelerating across one volt of electrical potential, and divide it by c2 getting eV/c2 or kilo eV/c2 (Kev/c2) or Million eV/c2 (Mev/c2) or even GeV/c2, a billion eV.
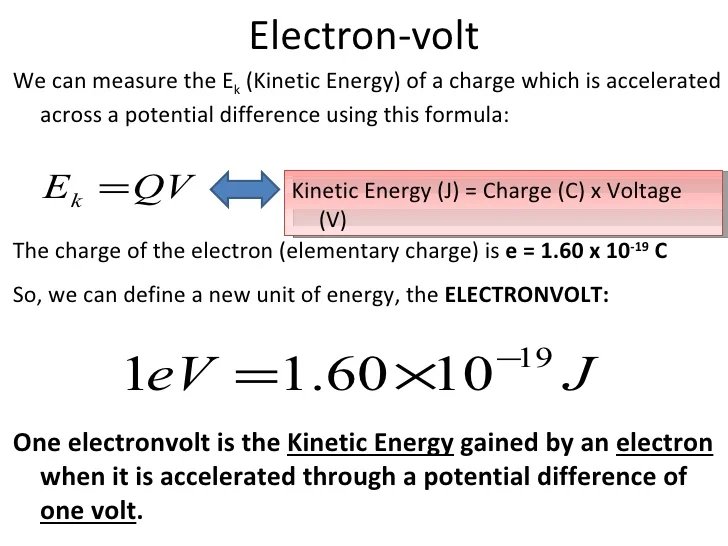
Now neutrons are themselves hard to handle, being neutral you can’t use an electric field to control them. So the KATRIN experiment uses the heavy isotope of hydrogen called Tritium, whose nuclei consists of one proton and two neutrons. Tritium is a well studied beta decay source and as a gas it is much easier to handle than a free neutron would be. Also the proton that results when the neutron decays remains in the nucleus, transforming it to a nucleus of helium-3. That means that the only thing you really have to measure is the energy of the produced electron.
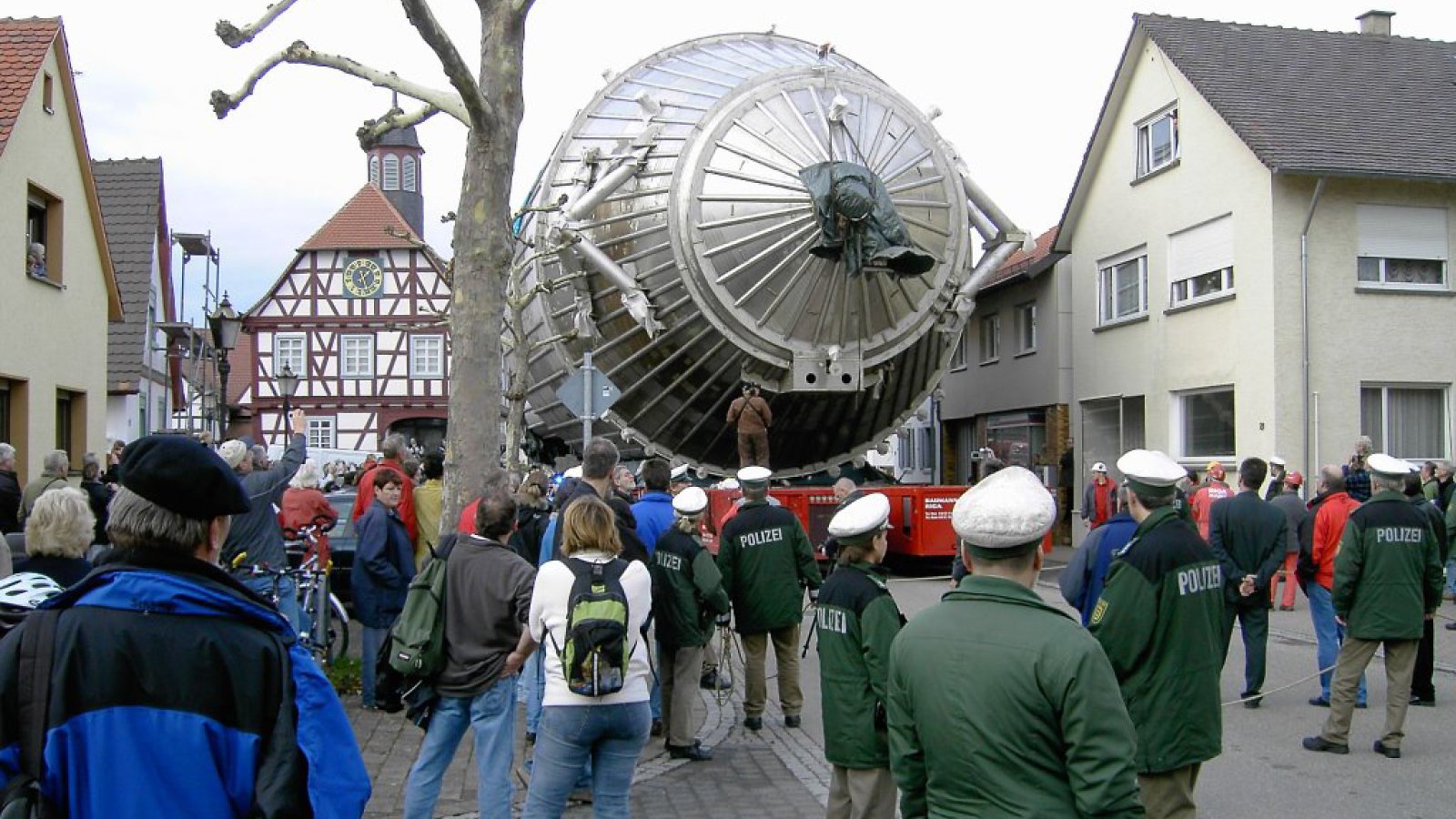
Nevertheless it’s still a difficult task, which is why the KATRIN experiment is an enormous instrument 70m in length, much of which is the main spectrometer for measuring the electron’s energy. For the experiment the tritium gas of cooled down to a temperature of 30K (-247ºC) in order to minimize thermal motion and an set of 24 superconducting magnets are used to collimate the emitted electrons into the spectrometer.
While KATRIN is still continuing to collect data an analysis of the measurements gathered by the end of 2019 has achieved a milestone, at the 90% confidence level the rest mass of a neutrino is less than 0.8 eV/c2. An elementary particle with a rest mass that is less than 1eV would have been a shocking result just a few decades ago and in a sense a rest mass of around one-millionth that of the electron, or even less, only deepens the mystery of elementary particle masses.
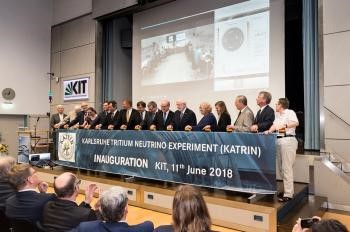
Still the results of KATRIN are reality and the only way to get beyond the standard model is to gather more facts that don’t fit in the model. Who knows, maybe right now some grad student in some university somewhere is reading the article published in Nature Physics by the KATRIN collaboration and is thinking to themselves, ‘hey, wait a minute… that actually makes sense’! After all, that’s how it started with Pauli, and Einstein, and Bohr and all those others who built the standard model.